
Wolf-Rayet Star
Joseph Wang, Rice Class of 2020
ASTR350 Final Project
Introduction
Wolf-Rayet stars are one stage on the evolution track of extremely massive stars. Spectroscopically, Wolf-Rayet stars are spectacular in appearance, with strong, broad emission lines instead of the narrow absorption lines that are typical of normal stellar populations. Their spectra are subsequently identified as those with strong lines of He and N (WN subtypes) and those with strong He, C, and O (WC and WO subtypes).
​
Wolf-Rayet stars typically have wind densities an order of magnitude higher than massive O stars. They contribute to the chemical enrichment of galaxies, they are the prime candidates for the immediate progenitors of long, soft gamma-ray bursts, and they provide a signature of high-mass star formation in galaxies.
​
The strong, broad emission lines seen in spectra of WR stars are due to their powerful stellar winds. The wind is sufficiently dense that an optical depth of unity in the continuum arises in the outflowing material. The spectral features are formed far out in the wind and are seen primarily in emission.
​
WR stars within the Milky Way are named in the order of right ascensions, starting from WR1, WR2 etc. Due to the rareness of these giants, with only around 300 discovered in our galaxy, such nomenclature works just fine.

Spectral Classification
Visual spectral classification of WR stars is based on emission line strengths and line ratios.
WN spectral subtypes follow a scheme involving line ratios of N iii-v and He i-ii, ranging from WN2 to WN5 for early WN (WNE) stars, and WN7 to WN9 for late WN (WNL) stars; WN6 stars can be either early or late type. A “h” suffix may be used to indicate the presence of emission lines due to H. The “ha” nomenclature indicates that H is seen both in absorption and emission lines. WNha stars show a continuity of properties between normal O stars and late-type WN stars. They are believed not to be the more mature, classic He-burning WN stars, but massive O stars with relative strong stellar winds. WNha stars are still in H core-burning stage!
​
WC spectral subtypes depend on the line ratios of C iii and C iv lines along with the appearance of O iii-v, spanning WC4 to WC9 subtypes, for which WC4–6 stars are early (WCE) and WC7–9 are late (WCL). Rare, O-rich WO stars form an extension of the WCE sequence, exhibiting strong O vi λ 3811–34 emission line.
​
Typical spectra for WN and WC stars are shown below.
![S5ICTJG68}W]070GD9`7B_E.png](https://static.wixstatic.com/media/81476c_e91adcd05aa24930a0a25a266d042ccc~mv2.png/v1/fill/w_608,h_688,al_c,q_90,usm_0.66_1.00_0.01,enc_avif,quality_auto/S5ICTJG68%7DW%5D070GD9%607B_E.png)
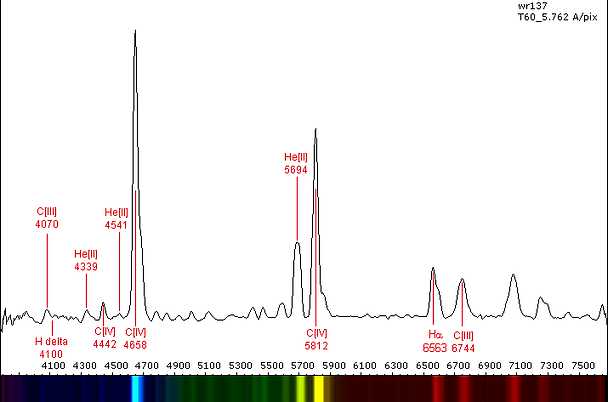
Spectrum of WR 137, a WC7 Star
Evolution
On the HR diagram, WR stars are sitting at the end of AGB phase, indicating that they are only one step from supernovae.
​
The WNL are the youngest Wolf-Rayet stars with an ongoing H-burning (shell burning). . As a consequence of development the WNE have now completed H-burning. He ii emission line gradually dominate as the CNO cycle fusing more and more Helium and He is carried to the atomsphere through convection.
In general, WN stars show products of CNO cycle and WC stars show products of triple-α process. It it natural to consider that WN stars can evolve to WC stars. A picture of current evolution model is shown below.
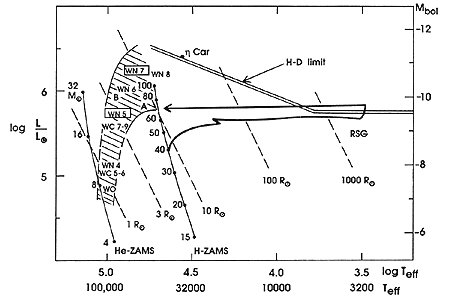
We notice that different subtypes of WR stars are actually mass dependent. The masses of the most evolved WC stars should be the smallest as they blow away most of their atmospheres. The youngest WNha stars still maintain most of their initial mass since they just start to expel their atmospheres.
​
The inital mass also affects the subtype of WR stars. Only the most massive O stars are able to evolve to WNha stage because of their extremely strong stellar winds, which ensure the presence of emission lines in their spectra even at H core-burning stage. Lower mass stars, however, may not have stellar winds strong enough to show the characteristics of WR stars before they becom supernovae.
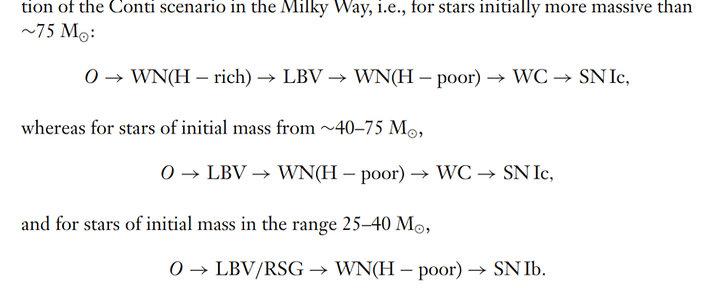
Absolute Magnitude/Luminosity
WR stars cannot be distinguished from normal hot stars using UBV photometry. Broad-band visual measurements overestimate the true continuum level in extreme cases by up to 1 mag, or more typically 0.5 mag for single early-type WR stars owing to their strong emission-line spectra. Consequently, astronomers developed a narrow band filter system, such that most photometry of WR stars has used the ubvr filter system, which is compared to broad band UBV system on the left.
​
Absolute visual magnitudes of WR stars are obtained primarily from calibrations obtained from cluster or association membership, since determination of WR distance via parallax is only possible for a few WR stars. Typical aboslute magnitudes range from Mv = –3 mag at earlier subtypes to –6 mag for late subtypes, or exceptionally –7 mag for H-rich WN stars. The trend in luminosities is of course similar to that in absolute magnitude. L(WNha) ~ 10^7 L⊙, L(WN) ~ 10^5.5 L⊙ and L(WC) ~ 10^5 L⊙. Even though WC stars are the hottest, the huge loss of mass (and associated shrinking of size) causes them to be the dimmest amongst WR stars.
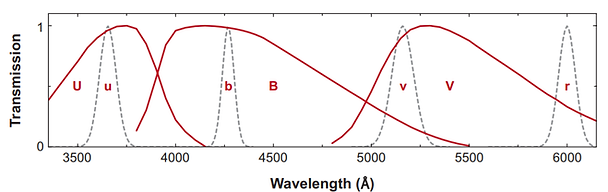
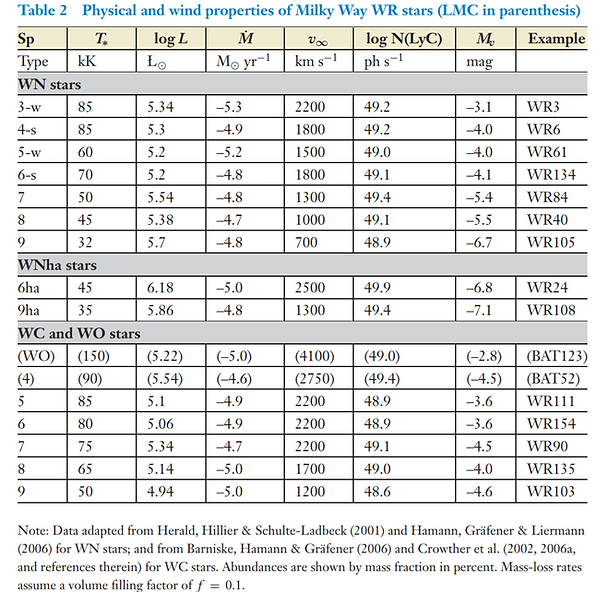
Distribution
WR stars are located in or close to massive star-forming regions within the Galactic disk. Although optical narrow-band surveys have proved very successful for identifying WR stars in the Solar Neighborhood, only a few hundred WR stars are known in the Milky Way, while many thousands are expected to exist within the Galactic disk, as mentioned before.
The annoying interstellar extinction prevents us from doing ultra high frequency survey, but switch to IR band survey. However, a limitation of IR emission-line surveys is that fluxes of near-IR lines are much weaker than those of optical lines. Also, no strong WR lines are common to all spectral types in the frequently used K band. Nevertheless, people are still trying to find the uniqueness of WR spectra in IR band, to implement IR band survey, as it is currently the only efficient way to discover WR stars within the Galactic disk.
​
It is well established that the absolute number of WR stars and their subtype distribution are metallicity dependent. N(WR)/N(O) ∼0.15 in the relatively metalrich Solar Neighborhood (Conti et al. 1983, van der Hucht 2001), yet N(WR)/N(O) ∼0.01 in the metal-deficient Small Magellanic Clouds (SMC) on the basis of only 12 WR stars (Massey, Olsen & Parker 2003) versus ∼1000 O stars (Evans et al. 2004).
Issues in Radiative Transfer
Our interpretation of hot, luminous stars via radiative transfer codes is hindered with respect to normal stars by several effects. First, the routine assumption of local thermodynamic equilibrium (LTE) breaks down for high-temperature stars. In non-LTE, the determination of populations uses rates that are functions of the radiation field, itself a function of the populations. Consequently, it is necessary to solve for the radiation field and populations iteratively. Second, the problem of accounting for the effect of millions of spectral lines upon the emergent atmospheric structure and emergent spectrum—known as line blanketing—remains challenging for stars in which spherical, rather than plane-parallel, geometry must be assumed owing to stellar winds, because the scale height of their atmospheres is not negligible with respect to their stellar radii. The combination of non-LTE, line blanketing (and availability of atomic data thereof ), and spherical geometry has prevented the routine analysis of such stars until recently. There are currently several methods dealing with these problems, but they are beyond the scope of this website.
LTE: Local Thermodynamic Eqilibrium
For a given region, if the distance for the temperature T to change significantly is much greater than the mean free path of particles in this region, we say this region reach local thermodynamic equilibrium, even it does not reach (global) thermodynamic equilibrium.
​
Blanketing Effect:
Blanketing effect is the enhancement of the red or infrared regions of a stellar spectrum at the expense of the other regions, with an overall diminishing effect on the whole spectrum. Astronomical metals, which produce most of a star's spectral absorption lines, absorb a fraction of the star's radiant energy (a phenomenon known as the blocking effect) and then re-emit it at a lower frequency as part of the backwarming effect. The combination of both these effects results in the position of stars in a color-color diagram to shift towards redder areas as the proportion of metals in them increases. The blanketing effect is thus highly dependent on the metallicity index of a star, which indicates the fraction of elements other than hydrogen and helium that compose it.
​
Stellar Temperature and Radii
Temperature
Stellar temperatures for WR stars are difficult to characterize, because their geometric extension is comparable with their stellar radii.
​
Stellar temperatures of WR stars are derived from lines from adjacent ionization stages of He or N for WN stars or lines of C for WC stars (ionization temperature, recalled the definition in class). Metals such as C, N, and O provide efficient coolants, such that the outer wind electron temperature is typically 8000 K to 12000 K. In addition, derived stellar temperatures depend sensitively upon the detailed inclusion of line-blanketing by Fe peak elements. Inferred bolometric corrections and stellar luminosities also depend upon detailed metal line-blanketing. However, the number of stars studied with models taking the issues in radiative transfer into account has been embarrassingly small. Only a limited number of WR stars have been studied in detail.
​
Radius
Atmospheric models for WR stars are typically parameterized by the radius of the inner boundary R∗ at high Rosseland optical depth τ_Ross ∼ 10. For a normal star, the photosphere (or the radius) is located at τ ~ 2/3, as shown in class. Typical WN and WC winds have reached a significant fraction of their terminal velocity before they become optically thin in the continuum. R_2/3, the radius at τRoss = 2/3, lies at highly supersonic velocities, well beyond the hydrostatic domain. For example, Crowther, Morris & Smith (2006b) obtain R∗ = 2.9 R⊙ and R_2/3 = 7.7 R⊙ for HD 50896 (WN4b), corresponding to T∗ = 85000 K and T2/3 = 52000 K, respectively.
​
Stellar structure models predict radii Revol that are significantly smaller than those derived from atmospheric models. For example, R∗ = 2.7 R⊙ for HD 191765 (WR134, WN6b), versus R_evol = 0.8 R⊙, which follows from hydrostatic evolutionary models. The discrepancy in hydrostatic radii between stellar structure and atmospheric models has not yet been resolved.

Stellar Wind
What makes WR stars so unique is due to their strong stellar winds. Unlike lower mass stars, stellar winds of which are driven by gas pressure, radiation pressure powers the stellar winds from WR stars. Thomson scattering dominates the continuum opacity in O and WR stars, while the basic mechanism by which their winds are driven is the transfer of photon momentum to the stellar atmosphere through the absorption by spectral lines.
​
Another important observation result is that stellar wind is metallicity dependent, with a empirical relation concluded from several papers(1).
​
A general idea is that the strength of stellar wind depends on the number of photons per second and the number of lines available for absorption, as inferred from the basic mechanism shown above. In particular, the photons with λ at the maximum of phtospheric flux would contribute the most to the momentum of the stellar atmosphere (photon with a wavelength which peaks at the blackboday radiation spectrum). This wavelength with maximum flux is undoubtedly temperature dependent, and this specific wavelength can correspond to some particular elements. Detailed explanations can be found in the following paper.
(Mokiem MR, De Koter A, Vink J, Puls J, Evans CJ, et al. 2007. Astron. Astrophys.)
​
​
(1)

Reference
Annu. Rev. Astron. Astrophys. 2007. 45:177–219 (Main source, any pictures I do not label the source comes from this article)
https://en.wikipedia.org/wiki/WR_124
https://en.wikipedia.org/wiki/Wolf%E2%80%93Rayet_star
https://www.eso.org/public/outreach/eduoff/cas/cas2003/casreports-2003/rep-142/Print_Version.htm
https://en.wikipedia.org/wiki/Blanketing_effect
Mokiem MR, De Koter A, Vink J, Puls J, Evans CJ, et al. 2007. Astron. Astrophys.